Demonstrating Method Suitability for an ATP-bioluminescence Rapid Microbial Detection Method for Biologic Products
As any industrial microbiologist is aware, all living cells contain ATP, and discerning microbial ATP from cellular ATP contents presents a challenge for developing test methodologies that require the detection of microbial ATP for interpreting a microbial contamination event. The following article will address this specifically in the application of testing biologic products and will also share cell line test data as examples of successful test methods.
As an example, consider the process of biologics manufacturing, mostly as it is related to harvest pools. The process becomes important when considering where microbial screening points become critical and how manufacturers can mitigate risk by implementing a real-time ATP-bioluminescence in-process test.
In the following illustration, biologics production starts with the cell bank and fermentation processes (also referred to as cell culture), where a small volume of a therapeutic protein-expressing cell line is pooled to larger and larger volumes, and thus, higher and higher cell densities. Typical cell lines used in biologics production are mammalian or avian, but can also be derived from transgenic plant or animal, insect, or bacterial/yeast origins.
Fig. 1: Biologics cell culture manufacturing illustration
As the initial working cell bank undergoes cell culture/fermentation and is transferred to larger and larger bioreactor vessels during scale up, the risk of introducing microbial contamination becomes both more likely and more costly. Additionally, it is common practice to scale up in individual smaller bioreactors in parallel, which are then pooled together into a larger vessel. The impact of a contamination here is also high, as a single contaminated vessel will still contaminate the entire pooled batch if not detected.
These microbial contaminants have been allowed to incubate alongside the therapeutic cell line. While potentially outcompeting the growth of the desired cells, reducing overall protein yield, microbial contaminants can also release toxins and waste products that contaminate the entire production batch. Given that additional downstream processes are optimized for controlled bioburden levels, they may not be optimized to purify or remove high contamination loads. It's important for manufacturers to not only prevent, but also rapidly detect contamination as early in the manufacturing process as possible to prevent it from spoiling an entire batch, resulting in wasted product, raw materials, characterized cell lines, and equipment cleaning.
In Figure 1 several cell pools are initially pooled into smaller ones. This is the ideal risk point for identifying bad pools, such that these can be discarded before they are combined into even larger batches. Unfortunately, manufacturers don't have a lot of time to wait, and so a microbiological screening test needs to be as fast as possible to find and remove the bad cell pools and move on to the next stage in the process.
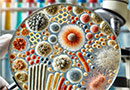
Recommendation
Barcelona, Spain23-25 September 2025
Modern Microbiology Laboratory
Some biologics manufacturers are using ATP-bioluminescence technology to test cell culture contamination in real time. There's no need for further incubation of samples because the cell culture sample has already incubated to serve target cell growth, serving in this case as the enrichment media itself. The challenge of this process is that samples contain high levels of background ATP from the target cell line, and any ATP-bioluminescence technology must successfully discern microbial ATP from background ATP presented by the sample.
Thinking outside the limitations of matching the capabilities of compendial microbial limits tests, it's important to understand that single cell detection is not the goal. Post-incubation, a contaminated cell line is likely to contain high levels of microbial bioburden, so a test sensitivity of around 1,000 CFU/ml is sufficient to serve its purpose when weighed against the risk of not discovering bad cell lines in real time. However, even with a limit of detection of around 1,000 CFU/ml, background ATP from the sample still has to be mitigated. Additionally, the test must be repeatable using different lots of cell lines so we know the test will be viable in various situations and production methods.
For this application, the ATP-bioluminescence technique analyzes liquid samples added to a cuvette. Figure 2 outlines the workflow for this test method. In the case of the in-process cell lines, this sample contains microbial contamination, which contains microbial ATP. The sample also contains non-microbial cells containing their own ATP as well as free ATP within the sample.
The intent of the detection reaction is to lyse the production cells and quench free cellular ATP, while leaving contaminant cells and their microbial ATP intact. In this Celsis® assay, a lysing reagent is added to samples to accomplish this objective. Next, an ATP-ase reagent hydrolyzes free ATP. After background ATP is removed or reduced from the sample, traditional ATP-bioluminescence techniques can be applied, and the Celsis® luminometer can detect light generated as a result of the ATP-bioluminescence reaction.
Fig. 2: An ATP-bioluminescence assay for in-process cell lines
Illustrated in Table 1 is an example of data generated during proof-of- concept tests where three different egg harvest pools used in viral replication were studied to understand background values of relative light units (or RLU's) generated when no microbial contaminants are present.
In this study, 30 individual datapoints were generated and averaged from each of three harvest pools containing different viral strains. These values were further evaluated to determine an appropriate pass-fail cutoff for future screening tests. Any sample reporting higher RLU values than the cutoff was considered to contain microbial contaminants. This can be set as deemed appropriate based on a risk assessment. The important point is to consider the method sensitivity desired for the in-process test.
Average RLU | RLU Baseline Established | |
Strain A | 345 | 533 |
Strain B | 285 | 789 |
Strain C | 433 | 659 |
Table 1: Average RLU baselines established for egg harvest pools
After baselines are established, the remaining egg harvest pools were tested using the ATP-bioluminescence technique described. To further demonstrate proof of concept, the strain illustrated was inoculated with varying known microbial loads and tested using ATP-bioluminescence to demonstrate signal to noise for contaminated samples. Proof-of-concept tests showed that for this lot, microbial bioburden greater than 1,000 CFU is easily discernable from the pass-fail cutoff previously established. Additionally, one can see that the data is highly linear - the greater the bioburden inoculated, the greater the RLU values reported (Reference Figure 3).
Fig. 3: RLU values for artifi cially contaminated egg pools
As further strains were evaluated, similar results were observed. One can see the results for a second and third strain that were studied as composited in Figure 4. Again, the data is highly linear and highly correlated to inocula levels, with significant separation between established sample baselines and inoculated samples.
The composite graph below offers another illustration as to the repeatability of this method across the various viral strains, demonstrating baselines and values for inoculated allantoic egg harvest pools. Ultimately, the data show that this real-time screening technology can be used to identify pooled allantoic harvest fluid and pooled cell culture harvests by extension, containing high levels of microbial contamination. This prevents problems in downstream filtration processes and minimizes the risk of discarding full batches during biologics production.
Fig. 4: Composite graph for RLU values for artifi cially contaminated cell lines
To summarize, the results were highly repeatable across varying viral strain types. Although the sensitivity of assays was high, reported as between 1x103 and 1x104 CFU/ml, the signal to noise ratios above the pass-fail cutoff were adequately significant to provide high reliability to the assay at these inocula levels. Through discussions with customers, Charles River found that this methodology has been adopted successfully for in-process testing of avian egg harvest pools, specifically for allantoic fluid pools.
Using ATP-bioluminescence technology to test pooled biologic samples at high method sensitivity is a good start. In fact, Charles River scientists were able to collaborate with cell manufacturers to produce a test method that reduces background ATP so that microbial contamination can be easily screened. However, in the greater context of a need for medicinal therapies, a test method that detects contamination levels upwards of 3-logs is certainly insufficient. The end goal is to develop a test method that produces a test sensitivity at or near the compendial sterility test.
Rapid Sterility Testing of Cell Containing Samples
When considering finished product sterility tests, the expectations are much stricter, as many industry professionals know. Although more recent guidance such as the recently released USP <1071> allow for a risk-based approach, it is still ideal to achieve at or near the test sensitivity of the compendial sterility test while also offering a rapid result. To achieve this, there is a need to further reduce background ATP levels without reducing signal from microbial ATP.
For this purpose, Charles River developed a new ATP-bioluminescence assay called Celsis Adapt™ Cell. This new assay has some additional steps which further reduce background ATP and considerably lowers method sensitivity. The first step of the assay is to perform the enrichment and incubation step as prescribed by the traditional sterility test. The test sample is typically added to about 100 ml of tryptic soy broth (TSB) and fluid thioglycollate (FTM). It is then incubated for 3-7 days. Next, the media are sampled and added to a treatment solution to aid in lysing the cellular component of the sample, while leaving microbial cells intact. Next, a physical treatment is performed using a Celsis Adapt™ sample concentrator, followed by application of an apyrase reagent, which was modified slightly for optimizing microbial detection. Finally, the Celsis® ATP assay is performed to screen for microbial contaminants. The addition of these method improvements further reduces the sample background and separation of signal from microbial ATP compared to the in-process test.
Figure 5 shows a comparison of the different ATP-bioluminescence assays that were tested. The first group of bar graphs represents samples tested using an amplified ATP-bioluminescence assay with none of the cellular treatment steps as outlined previously. Negative media controls are represented in blue and orange, showing RLU values of around 1000 RLU. When samples containing cells (in this example CHO cells) were added to the media, RLU values were upwards of 100,000 RLU. Certainly, microbial ATP would not be discernable from a sample tested in this manner.
When an apyrase reagent was added, the test results do not change dramatically for this cell line. In fact, media negative control signals lowered slightly, but the sample signal did not appear to be reduced. Finally, when the additional optimized cell treatment was applied, there was a dramatic decrease in RLU from cellular ATP in the sample. There was some elevation of RLU, but the dramatic decrease allowed the assay to discern microbial ATP when present at levels above the baseline noise of the sample.
Fig. 5: Background of 10% cell samples with diff erent treatments
The study then evaluated the assay's ability to detect microbial ATP when the optimized cell assay is performed. The results are shown in Figure 6. For this series of tests, Charles River looked at a few different microorganisms, including fungal species, taking into account different incubation temperatures or even media in an eff ort to better characterize the new assay method. Each bar on the graph represents each subsequent day that the incubated media was read using the Celsis® ATP assay. Whenever two subsequent positive assays were measured, the incubation was ended for that challenge microorganism.
When evaluating Bacillus subtilis, one can see that B. subtilis prefers 35 °C incubation over 25 °C since B. subtilis was detected after just one day of incubation at 35 °C and aft er two days at 25 °C. As depicted in Figure 6, Aspergillus brasiliensis is also successfully detected using the new cell assay in just three days. Finally, the study examined a slow grower, Cutibacterium acnes, as it is known to be one of the greatest challenges for ATP-bioluminescence assays.
Fig. 6: Time to detect
For this assay, an arbitrary pass-fail cutoff at 5000 RLU was set since the backgrounds of various cell types haven't yet been fully characterized. The results of the graph, representing tests performed without any product, show that microbial cultures of < 10 CFU all generated positive results in about three days.
After demonstrating an assay that reduces cellular ATP and allows for detection of microbial ATP, Charles River performed tests in the presence of both in order to further demonstrate the application of the improved methodology. Two cell lines were selected for the following experiments, labeled as "Cell Line 1" and "Cell Line 2".
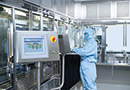
Recommendation
Cologne, Germany21-23 October 2025
Lyophilization 2025 - Includes Workshop at GEA
As mentioned previously, the new assay requires an enrichment and incubation step, unlike the in-process assay discussed previously. The compendial assay method already in place was used, but instead of checking for visual turbidity, the new Celsis® assay was used to determine results. In this case, methods used direct inoculation of TSB and FTM medias, as would often be the case for cellbased preparations since membrane filtration has historically been observed to be problematic.
In this example, two cell lines were tested using two direct inoculation methods, in which the concentration of cells per volume of media was higher for Method 2 (in red) than for Method 1 (in blue).
Fig. 7: Microbial recovery from cell samples in FTM
Note that the charts in Figure 7 are based on a logarithmic scale. Media controls reported RLU values in the range of two logs (128 RLU), and cell samples themselves in the range of about three. When inoculated with Staphylococcus aureus pre-incubation, significantly elevated RLU values were found at the end of incubation in the range of six or even seven logs.
The key point of the data is the difference between RLU values reported for non-contaminated cell samples versus samples contaminated with a low level of challenge microorganism. Signal-to-noise ratios demonstrate that, on average, when inoculated with only 38 CFU of S. aureus, results for Cell Line 1 were 23,000 times higher than controls and results for Cell Line 2 were 1,900 times higher than controls. One should keep in mind that even a log increase in RLU value is considered significant for the purposes of discerning microbial contamination from background signal. Similar values were also reported for Clostridium sporogenes.
Fig. 8: Microbial recovery from cell samples in TSB
As a final example for this study, Figure 8 represents results for tests performed using the challenge microorganism A. brasiliensis. There are some limitations commonly known to rapid methods when it comes to mold detection. Through this study, this revised cell application method led to vast improvements in mold detection over other non-ATP based rapid methods.
In conclusion, results from the Celsis Adapt™ Cell assay demonstrated that at low microbial levels, specifically using inoculums of less than 100 CFU or even 10 CFU, the assay successfully detects microbial contaminants in less than half the time of a traditional sterility test.
High signal-to-noise ratios demonstrate that even at low levels, a high separation value exists, facilitating the interpretation of a positive result from a negative one in just a few days. Additionally, tests demonstrated specificity, demonstrating the ATP assay is equally capable of detecting bacterial and fungal species.
Following the success of these tests using the cell lines described in this article, Charles River has received and tested many various additional cell lines with continued success with over 20 cell lines. The data continue to demonstrate wide applicability of the assay, and the assay has yet to encounter problematic or incompatible cell lines thus far.
The greatest challenge to developing an adequate sterility test is overcoming inhibition, which is a problem even for a traditional sterility test. This demonstrates that when microbial contaminants are present, such that they are not inhibited by the sample, our ATP-bioluminescence assay can detect them.
About the Author:
Stacey Ramsey (MS)
... originally joined Charles River as an associate product manager and currently manages the Celsis® Technical Services and Validations laboratory based in Charleston, South Carolina, USA.